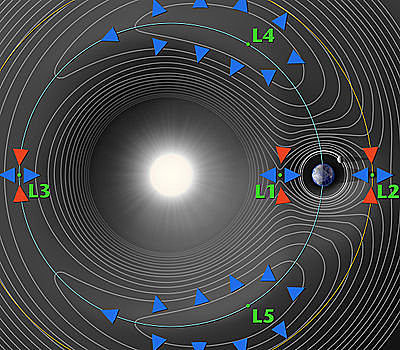
http://zh.wikipedia.org/wiki/%E6%8B%89%E6%A0%BC%E6%9C%97%E6%97%A5%E7%82%B9
http://en.wikipedia.org/wiki/Lagrangian_point
The Lagrangian points ( /ləˈɡrɑːndʒiən/; also Lagrange points, L-points, or libration points) are the five positions in an orbital configuration where a small object affected only by gravity can theoretically be stationary relative to two larger objects (such as a satellite with respect to the Earth and Moon). The Lagrange points mark positions where the combined gravitational pull of the two large masses provides precisely the centripetal force required to rotate with them. They are analogous to geostationary orbits in that they allow an object to be in a fixed position relative to a body (or indeed, two bodies) rather than any more general orbit in which its relative position changes continuously.
Lagrangian points are the stationary solutions of the circular restricted three-body problem. For example, given two massive bodies in circular orbits around their common center of mass, there are five positions in space where a third body, of comparatively negligible mass, could be placed which would then maintain its position relative to the two massive bodies. As seen in a rotating reference frame with the same period as the two co-orbiting bodies, the gravitational fields of two massive bodies combined with the satellite's circular motion are in balance at the Lagrangian points, allowing the third body to be stationary with respect to the first two bodies.[1]
The three collinear Lagrange points were first discovered by Leonhard Euler around 1750.[2]
In 1772, the Italian-French mathematician Joseph Louis Lagrange was working on the famous three-body problem when he discovered an interesting quirk in the results. Originally, he had set out to discover a way to easily calculate the gravitational interaction between arbitrary numbers of bodies in a system, because Newtonian mechanics concludes that such a system results in the bodies orbiting chaotically until there is a collision, or a body is thrown out of the system so that equilibrium can be achieved.
The logic behind this conclusion is that a system with one body is trivial, as it is merely static relative to itself; a system with two bodies is the relatively simple two-body problem, with the bodies orbiting around their common center of mass. However, once more than two bodies are introduced, the mathematical calculations become very complicated. It becomes necessary to calculate the gravitational interaction between every pair of objects at every point along its trajectory.
Lagrange, however, wanted to make this simpler. He did so with a simple hypothesis: The trajectory of an object is determined by finding a path that minimizes the action over time. This is found by subtracting the potential energy from the kinetic energy. With this way of thinking, Lagrange re-formulated the classical Newtonian mechanics to give rise to Lagrangian mechanics. With his new system of calculations, Lagrange’s work led him to hypothesize how a third body of negligible mass would orbit around two larger bodies which were already in a near-circular orbit. In a frame of reference that rotates with the larger bodies, he found five specific fixed points where the third body experiences zero net force as it follows the circular orbit of its host bodies (planets).[3] These points were named “Lagrangian points” in Lagrange's honor. It took over a hundred years before his mathematical theory was validated by the discovery of the Trojan asteroids at the L4 and L5 Lagrange points of the Sun–Jupiter system in 1906.
In the more general case of elliptical orbits, there are no longer stationary points in the same sense: it becomes more of a Lagrangian “area”. The Lagrangian points constructed at each point in time, as in the circular case, form stationary elliptical orbits which are similar to the orbits of the massive bodies. This is due to Newton's second law (Force = Mass times Acceleration, or ), where p = mv (p the momentum, m the mass, and v the velocity) is invariant if force and position are scaled by the same factor. A body at a Lagrangian point orbits with the same period as the two massive bodies in the circular case, implying that it has the same ratio of gravitational force to radial distance as they do. This fact is independent of the circularity of the orbits, and it implies that the elliptical orbits traced by the Lagrangian points are solutions of the equation of motion of the third body.[citation needed]
The Lagrangian points
The five Lagrangian points are labeled and defined as follows:
[edit] L1
The L1 point lies on the line defined by the two large masses M1 and M2, and between them. It is the most intuitively understood of the Lagrangian points: the one where the gravitational attraction of M2 partially cancels M1 gravitational attraction. It is the only L-point which exists in non-rotating systems.
- Example: An object which orbits the Sun more closely than the Earth would normally have a shorter orbital period than the Earth, but that ignores the effect of the Earth's own gravitational pull. If the object is directly between the Earth and the Sun, then the effect of the Earth's gravity is to weaken the force pulling the object towards the Sun, and therefore increase the orbital period of the object. The closer to Earth the object is, the greater this effect is. At the L1 point, the orbital period of the object becomes exactly equal to the Earth's orbital period. L1 is about 1.5 million kilometers from the Earth. Gravity from the Sun is 2% (118 µm/s²) more than at the Earth's orbit (5.9 mm/s²), while the reduction of required centripetal force is half of this (59 µm/s²). The sum of both effects is balanced by the gravity of the Earth, which is here also 177 µm/s².[citation needed]
The Sun–Earth L1 is suited for making observations of the Sun. Objects here are never shadowed by the Earth or the Moon. The Solar and Heliospheric Observatory (SOHO) is stationed in a Halo orbit at L1, and the Advanced Composition Explorer (ACE) is in a Lissajous orbit, also at the L1 point. WIND is also at L1.
The Earth–Moon L1 allows comparatively easy access to lunar and earth orbits with minimal change in velocity and has this as an advantage to position a half-way manned space station intended to help transport cargo and personnel to the Moon and back.
[edit] L2
The L2 point lies on the line defined by the two large masses, beyond the smaller of the two. Here, the gravitational forces of the two large masses balance the centrifugal effect on the smaller mass.
- Example: On the side of the Earth away from the Sun, the orbital period of an object would normally be greater than that of the Earth. The extra pull of the Earth's gravity decreases the orbital period of the object, and at the L2 point that orbital period becomes equal to the Earth's.
The Sun-Earth L2 is a good spot for space-based observatories. Because an object around L2 will maintain the same orientation with respect to the Sun and Earth, shielding and calibration are much simpler. It is, however, slightly beyond the reach of Earth's umbra,[4] so solar radiation is not completely blocked. The Wilkinson Microwave Anisotropy Probe, Herschel Space Observatory and Planck space observatory are already in orbit around the Sun-Earth L2. The Gaia probe and James Webb Space Telescope will be placed at the Sun-Earth L2. Earth-Moon L2 would be a good location for a communications satellite covering the Moon's far side. Earth-Moon L2 would be "an ideal location" for a propellant depot as part of the proposed Depot-based space transportation architecture.[5]
If the mass of the smaller object (M2) is much smaller than the mass of the larger object (M1) then L1 and L2 are at approximately equal distances r from the smaller object, equal to the radius of the Hill sphere, given by:
where R is the distance between the two bodies.
This distance can be described as being such that the orbital period, corresponding to a circular orbit with this distance as radius around M2 in the absence of M1, is that of M2 around M1, divided by :
[edit] Examples
- Sun and Earth: 1,500,000 km (930,000 mi) from the Earth
- Earth and Moon: 61,500 km (38,200 mi) from the Moon
[edit] L3
The L3 point lies on the line defined by the two large masses, beyond the larger of the two.
- Example: L3 in the Sun–Earth system exists on the opposite side of the Sun, a little outside the Earth's orbit but slightly closer to the Sun than the Earth is. (This apparent contradiction is because the Sun is also affected by the Earth's gravity, and so orbits around the two bodies' barycenter, which is however well inside the body of the Sun.) At the L3 point, the combined pull of the Earth and Sun again causes the object to orbit with the same period as the Earth.
The Sun–Earth L3 point was a popular place to put a "Counter-Earth" in pulp science fiction and comic books. Once space-based observation became possible via satellites and probes, it was shown to hold no such object. The Sun–Earth L3 is unstable and could not contain an object, large or small, for very long. This is because the gravitational forces of the other planets are stronger than that of the Earth (Venus, for example, comes within 0.3 AU of this L3 every 20 months). In addition, because Earth's orbit is elliptical and because the barycenter of the Sun-Jupiter system is unbalanced relative to Earth, such a Counter-Earth would frequently be visible from Earth.
A spacecraft orbiting near Sun-Earth L3 would be able to closely monitor the evolution of active sunspot regions before they rotate into a geoeffective position, so that a 7-day early warning could be issued by the NOAA Space Weather Prediction Center. Moreover, a satellite near Sun-Earth L3 would provide very important observations not only for Earth forecasts, but also for deep space support (Mars predictions and for manned mission to near-Earth asteroids). In 2010, spacecraft transfer trajectories to Sun-Earth L3 were studied and several designs were considered.[6]
One example of asteroids which visit an L3 point is the Hilda family whose orbit brings them to the Sun-Jupiter L3 point.
[edit] L4 and L5
The L4 and L5 points lie at the third corners of the two equilateral triangles in the plane of orbit whose common base is the line between the centers of the two masses, such that the point lies behind (L5) or ahead of (L4) the smaller mass with regard to its orbit around the larger mass.
The reason these points are in balance is that, at L4 and L5, the distances to the two masses are equal. Accordingly, the gravitational forces from the two massive bodies are in the same ratio as the masses of the two bodies, and so the resultant force acts through the barycenter of the system; additionally, the geometry of the triangle ensures that the resultant acceleration is to the distance from the barycenter in the same ratio as for the two massive bodies. The barycenter being both the center of mass and center of rotation of the system, this resultant force is exactly that required to keep a body at the Lagrange point in orbital equilibrium with the rest of the system. (Indeed, the third body need not have negligible mass). The general triangular configuration was discovered by Lagrange in work on the 3-body problem.
L4 and L5 are sometimes called triangular Lagrange points or Trojan points. The name Trojan points comes from the Trojan asteroids at the Sun–Jupiter L4 and L5 points, which themselves are named after characters from Homer's Iliad (the legendary siege of Troy). Asteroids at the L4 point, which leads Jupiter, are referred to as the "Greek camp", while at the L5 point they are referred to as the "Trojan camp". These asteroids are (largely) named after characters from the respective sides of the Trojan War.
[edit] Examples
- The Sun–Earth L4 and L5 points lie 60° ahead of and 60° behind the Earth as it orbits the Sun. They contain interplanetary dust.
- The Earth–Moon L4 and L5 points lie 60° ahead of and 60° behind the Moon as it orbits the Earth. They may contain interplanetary dust in what is called Kordylewski clouds.
- The Sun–Jupiter L4 and L5 points are occupied by the Trojan asteroids.
- The Sun-Neptune L4 and L5 points have Trojan objects.[7]
- Saturn's moon Tethys has two much smaller satellites at its L4 and L5 points named Telesto and Calypso, respectively.
- Saturn's moon Dione has smaller moons Helene and Polydeuces at its L4 and L5 points, respectively.
- One version of the giant impact hypothesis suggests that an object named Theia formed at the Sun–Earth L4 or L5 points and crashed into the Earth after its orbit destabilized, forming the moon.